Today was a very good day to visit the Zurich rainforest. Although the leaf-tailed gecko pictures didn’t turn out so well, I caught a no-tailed gecko
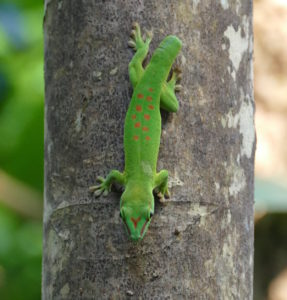
and a fruit-eating gecko
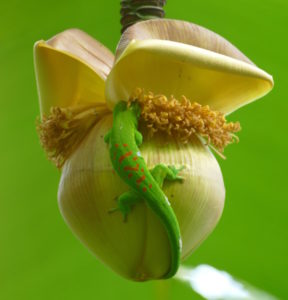
and the shadow of a bird…
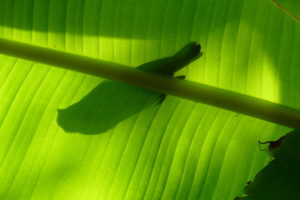
Today was a very good day to visit the Zurich rainforest. Although the leaf-tailed gecko pictures didn’t turn out so well, I caught a no-tailed gecko
and a fruit-eating gecko
and the shadow of a bird…
It’s not every day that I have three upcoming conferences to announce which I co-organize. In chronological order:
June 1 and 2, 2018, Ph. Habegger, Ph. Michel and myself are organizing the 14th edition of the “Number Theory Days”. It will be in Basel, for the first time — from then on, it will rotate between Basel, Lausanne and Zürich.
January 22 to 25, 2019, C. Burrin, T. Hartnick, B. Pozzeti, A. Wienhard and myself and co-organizing a conference at FIM in honor of the birthday of A. Iozzi.
June 17 to 22, 2019, Ö. Imamoglu, H. Iwaniec and myself are co-organizing a conference at FIM in honor of the birthday of B. Duke.
The respective web pages have more information (and in the last two cases, these will be updated when the FIM webpage is created; this will contain the registration form, as well as the web page to request funding for junior participants).
Subsidiary question: Which book is Bill Duke holding in the picture? Any correct answer (without cheating) gives right to one drink of your choice.
When, exactly two years ago, I published my earlier post containing the story of J. Ménard, I was apparently suspected by some people of being the author of that text. I tried for a long time to find the original Spanish version mentioned in the text, whose existence conclusively refutes this assertion (since my understanding of Spanish is, unfortunately, non-existent). After much effort, I have finally succeeded!
This afternoon, while chatting with Will Sawin, between addressing rather technical points of ongoing projects, we observed that although we’ve seen seminar talks shared between two speakers, it was never with simultaneous speakers. It was just a step to jump from there to the idea that someone should write an Opera about a mathematical seminar talk, which — as opera does — would allow a duet, or trio, or quartet, or quintet, or sextet, of simultaneous speakers, including maybe some from the audience, or the chairperson trying to control the situation.
Unfortunately, I don’t know music, but if I were twenty years old, I’d be very tempted to write “Seminar”, the definitive opera about a math talk. At least, I can think of a libretto and try to write it (which language? I think French is best here, although the title should then be “Séminaire” or “L’exposé” in that case; which topic? good question — of course it would have to be a real talk; which style?)
In any case, I can safely predict a triumph in enlightened circles.
I already discussed the very interesting mathematics related to the Bohr-Pál Theorem that I learnt about while writing my preprint with W. Sawin on the support of Kloosterman path. This also led us (very) tangentially to what turns out to be an open geometric problem: Mihalik and Wieczorek asked whether there exists a continuous function which sends every interval in
to a convex subset of
, and whose image is not contained in an affine line in
. In other words: suppose
is continuous and satisfies the intermediate value property (in the sense that for any
, any point on the segment between
and
is of the form
for some
between
and
); is the image of
contained in some affine line?
The existence of such functions may seem unlikely, but experience shows that being unlikely to exist has rarely stopped functions from actually existing.
The arithmetic relevance of a hypothetical Mihalik-Wieczorek function is that, by adapting Sahakyan’s argument (as discussed before), it seems that we would be able to construct a function in the support of Kloosterman paths that has image containing an open set, answering one of our lingering questions.
However, the question remains apparently open. The best known result (that we know about) is due to Pach and Rogers (1982), and independently Vince and Wilson (1984): there exists , with image not contained in an affine line, such that
and
are convex for all
. (Note that Vince and Wilson conjecture at the end of their paper that a Mihalik-Wieczorek curve does not exist; however their argument is based on a weaker conjecture that this existence would imply, and that statement in turn is actually true, as was explicitly stated by Pach and Rogers at the end of their article; see this American Math. Monthly problem.).
Update (May 13, 2018) I’ve written a short note that checks in detail the statement above: if a Mihalik-Wieczorek curve exists, then there exists a space-filling curve in the support of the Kloosterman paths. (More precisely, this is done without the symmetry requirement of the Kloosterman paths, but these can be ensured by scaling the given curve to and then reflecting it on
). This note can also be seen as a detailed account of Sahakian’s Theorem…